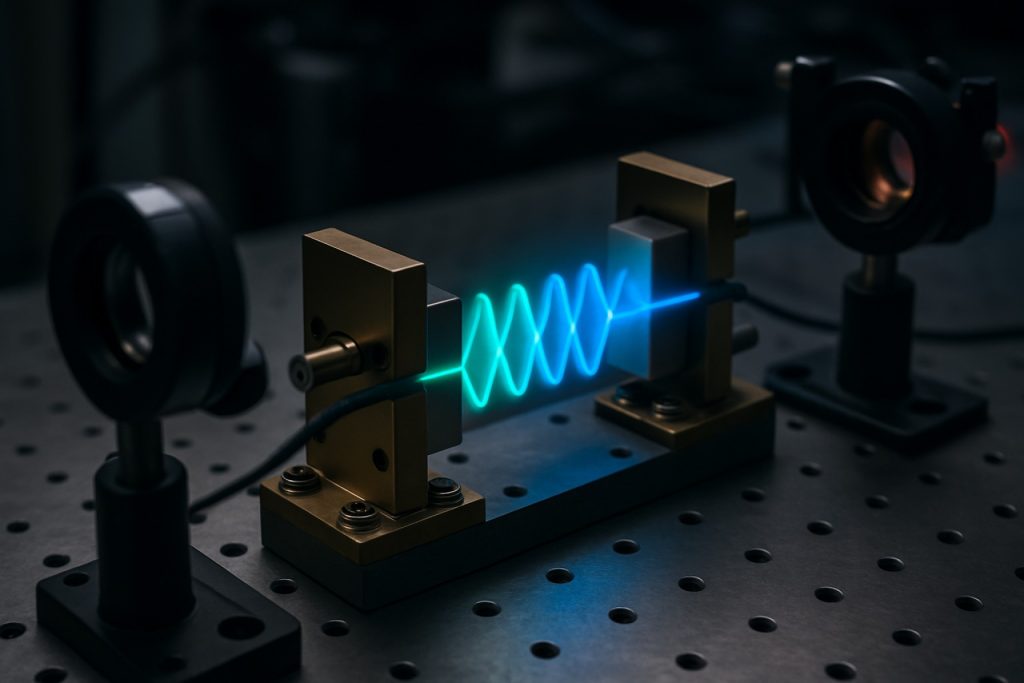
Backward Wave Coupling Explained: How Counterintuitive Energy Flows Are Transforming Photonics and Signal Processing. Discover the Science Behind This Groundbreaking Phenomenon.
- Introduction to Backward Wave Coupling
- Historical Development and Key Discoveries
- Fundamental Physics: Understanding Wave Propagation
- Mathematical Models and Theoretical Frameworks
- Experimental Techniques and Measurement Methods
- Backward Wave Coupling in Photonic Devices
- Applications in Telecommunications and Signal Processing
- Challenges and Limitations in Practical Implementations
- Recent Advances and Emerging Research Trends
- Future Prospects and Potential Technological Impacts
- Sources & References
Introduction to Backward Wave Coupling
Backward wave coupling is a fundamental phenomenon in the field of wave physics, particularly relevant to the study of electromagnetic and acoustic wave propagation in engineered structures. Unlike conventional forward wave propagation, where the phase and energy of a wave travel in the same direction, backward wave coupling occurs when the phase velocity and group velocity of a wave are antiparallel—meaning the energy flows in the opposite direction to the phase front. This counterintuitive behavior is most commonly observed in periodic media, such as photonic crystals, metamaterials, and certain types of waveguides.
The concept of backward wave propagation was first explored in the context of microwave engineering and later extended to optics and acoustics. In these systems, backward wave coupling can be engineered by introducing periodic variations in the material’s refractive index or acoustic impedance, leading to the formation of bandgaps and negative dispersion regions. In such regions, the interaction between forward and backward propagating modes enables unique effects, including negative refraction, reverse Cherenkov radiation, and the possibility of compact, highly efficient devices for filtering, amplification, and frequency conversion.
One of the most prominent applications of backward wave coupling is in the design of IEEE-standardized traveling wave tubes (TWTs) and backward wave oscillators (BWOs), which are critical components in high-frequency microwave and millimeter-wave systems. In these devices, the coupling between an electron beam and a backward electromagnetic wave enables efficient energy transfer and amplification, making them indispensable in radar, satellite communications, and scientific instrumentation.
In the realm of optics, backward wave coupling underpins the operation of distributed feedback (DFB) lasers and Bragg gratings, where periodic structures induce strong coupling between forward and backward propagating light waves. This leads to narrow linewidths and stable single-mode operation, which are essential for high-precision optical communication systems. Research institutions such as National Institute of Standards and Technology (NIST) and Optica (formerly Optical Society of America) have contributed significantly to the theoretical and experimental understanding of backward wave phenomena in photonic devices.
Overall, backward wave coupling represents a cornerstone of modern wave-based technology, enabling novel device architectures and functionalities that are unattainable with conventional forward wave propagation. Its study continues to drive innovation in fields ranging from telecommunications to quantum information science.
Historical Development and Key Discoveries
The concept of backward wave coupling has its roots in the early investigations of wave propagation in periodic structures, a field that gained momentum in the mid-20th century. The phenomenon was first observed in the context of microwave engineering, particularly within the study of traveling-wave tubes (TWTs) and backward wave oscillators (BWOs). These devices, which rely on the interaction between an electron beam and a slow-wave structure, demonstrated that under certain conditions, the phase velocity of the electromagnetic wave could be directed opposite to the energy flow, giving rise to what is now termed a “backward wave.”
A pivotal moment in the historical development of backward wave coupling occurred in the 1950s, when researchers at institutions such as the National Aeronautics and Space Administration (NASA) and the Institute of Electrical and Electronics Engineers (IEEE) began systematically studying the properties of slow-wave structures. The discovery that periodic metallic or dielectric structures could support backward waves led to the design of more efficient microwave amplifiers and oscillators. The theoretical framework for these phenomena was further solidified by the work of Soviet and American physicists, who developed mathematical models describing the coupling mechanisms between forward and backward propagating waves in periodic media.
The 1960s and 1970s saw the extension of backward wave concepts into the field of nonlinear optics. Researchers at the Optica (formerly Optical Society of America) and leading academic institutions explored how nonlinear interactions in crystals could generate backward-propagating waves, a principle that underpins modern parametric oscillators and frequency converters. The realization that backward wave coupling could be harnessed for efficient energy transfer and frequency conversion marked a significant milestone in photonics.
In the late 20th and early 21st centuries, the advent of metamaterials—engineered materials with tailored electromagnetic properties—reinvigorated interest in backward wave phenomena. Organizations such as the National Institute of Standards and Technology (NIST) played a crucial role in characterizing the unique dispersion properties of these materials, which can exhibit negative refractive indices and support backward wave propagation. This breakthrough has opened new avenues for applications in superlensing, cloaking, and advanced signal processing.
Today, backward wave coupling remains a vibrant area of research, with ongoing contributions from international standards bodies, academic consortia, and government laboratories. The historical trajectory of this field underscores the interplay between theoretical insights and technological innovation, driving advancements in both fundamental science and practical engineering.
Fundamental Physics: Understanding Wave Propagation
Backward wave coupling is a phenomenon in wave physics where the direction of energy flow (group velocity) is opposite to the direction of the phase velocity. This counterintuitive behavior is most commonly observed in engineered materials such as negative-index metamaterials and certain types of photonic crystals. In conventional media, both the phase and group velocities of a wave typically point in the same direction, meaning energy and wavefronts propagate together. However, in backward wave media, the phase fronts move in one direction while the energy propagates in the opposite direction, leading to unique electromagnetic properties.
The fundamental physics underlying backward wave coupling is rooted in the material’s electromagnetic response, specifically its permittivity and permeability. When both these parameters are negative over a certain frequency range, the material exhibits a negative refractive index. This was first theorized by Victor Veselago in the 1960s, who predicted that such materials would support backward waves, resulting in reversed Snell’s law, reversed Doppler effect, and reversed Cherenkov radiation. These predictions were experimentally realized decades later with the advent of metamaterials—artificially structured composites engineered to achieve electromagnetic properties not found in nature. Organizations such as the National Institute of Standards and Technology (NIST) and the Oak Ridge National Laboratory (ORNL) have contributed to the development and characterization of these materials.
Backward wave coupling is not limited to electromagnetic waves; it can also occur in acoustic and elastic wave systems. In photonic and phononic crystals, periodic structuring at the subwavelength scale can give rise to band structures where backward wave propagation is allowed. This is characterized by a negative slope in the dispersion relation, indicating that the group velocity (energy flow) is antiparallel to the phase velocity. Such behavior enables novel device concepts, including superlenses that surpass the diffraction limit and compact, nonreciprocal waveguides.
The study of backward wave coupling has significant implications for fundamental physics and practical applications. It challenges conventional understanding of wave propagation and enables the design of devices with unprecedented control over light and sound. Research in this area is ongoing at leading institutions such as the Nature Publishing Group (which publishes peer-reviewed studies in this field) and is supported by international standards bodies like the International Electrotechnical Commission (IEC), which develops standards for electromagnetic compatibility and material properties.
Mathematical Models and Theoretical Frameworks
Backward wave coupling is a phenomenon in wave physics where energy transfer occurs between waves propagating in opposite directions, often within engineered structures such as photonic crystals, metamaterials, or periodic waveguides. The mathematical modeling of backward wave coupling is essential for understanding and designing devices that exploit this effect, such as distributed feedback lasers, Bragg gratings, and negative-index materials.
The theoretical framework for backward wave coupling is typically grounded in coupled-mode theory (CMT), which provides a set of differential equations describing the interaction between forward and backward propagating modes. In its simplest form, the coupled-mode equations for the amplitudes (A(z)) (forward) and (B(z)) (backward) along the propagation direction (z) are:
- (frac{dA}{dz} = ikappa B e^{i2Delta kz})
- (frac{dB}{dz} = ikappa^* A e^{-i2Delta kz})
Here, (kappa) is the coupling coefficient, and (Delta k) is the phase mismatch between the interacting waves. These equations capture the essence of energy exchange due to periodic perturbations in the medium, such as refractive index modulations in fiber Bragg gratings or periodic loading in microwave transmission lines.
In the context of metamaterials, backward wave coupling is closely related to the existence of negative group velocity modes, where the phase and energy flow in opposite directions. Theoretical models for such materials often employ effective medium theory and Bloch-Floquet analysis to derive dispersion relations that predict backward wave propagation. The American Physical Society and IEEE have published foundational research on these topics, providing rigorous mathematical treatments and experimental validations.
For photonic crystals and periodic structures, the transfer matrix method and Bloch theorem are frequently used to analyze backward wave coupling. These approaches allow for the calculation of band structures and reflection/transmission spectra, revealing stop bands and resonance conditions where backward coupling is maximized. The Optica (formerly Optical Society of America) has been instrumental in disseminating research on photonic bandgap materials and their coupling phenomena.
Overall, the mathematical models and theoretical frameworks for backward wave coupling integrate concepts from electromagnetics, solid-state physics, and nonlinear optics. They provide predictive power for the design of advanced photonic and microwave devices, enabling innovations in communication, sensing, and signal processing technologies.
Experimental Techniques and Measurement Methods
Backward wave coupling is a phenomenon observed in various waveguide and resonator systems, where the energy transfer occurs in a direction opposite to the incident wave propagation. This effect is particularly significant in the study of photonic crystals, metamaterials, and certain types of microwave and optical devices. Experimental investigation of backward wave coupling requires precise techniques to both generate and detect the backward-propagating modes, as well as to distinguish them from forward-propagating or spurious signals.
A common experimental setup involves the use of directional couplers or circulators, which are devices designed to separate forward and backward traveling waves within a waveguide or fiber. By injecting a known signal into the system and monitoring the output at various ports, researchers can directly measure the amplitude and phase of the backward-coupled wave. The use of National Institute of Standards and Technology (NIST)-calibrated vector network analyzers (VNAs) is standard practice for characterizing the scattering parameters (S-parameters) that describe the coupling efficiency and directionality.
In optical systems, backward wave coupling is often studied using fiber Bragg gratings or photonic crystal waveguides. Here, a tunable laser source is used to launch light into the structure, and the reflected (backward-coupled) signal is measured with high-sensitivity photodetectors. The spectral response is analyzed to determine the coupling strength and bandwidth. For experiments at microwave or terahertz frequencies, researchers may employ time-domain reflectometry or frequency-domain techniques, using pulsed or continuous-wave sources, respectively.
Advanced measurement methods include near-field scanning techniques, where a probe is scanned in close proximity to the waveguide or resonator to map the spatial distribution of the electromagnetic fields. This allows for direct visualization of the backward wave and its interaction with the structure. Cryogenic setups are sometimes used to reduce thermal noise and enhance measurement sensitivity, especially in superconducting or quantum systems.
Calibration and error analysis are critical in these experiments, as backward wave signals are often much weaker than their forward counterparts. Standard reference materials and procedures, such as those developed by International Electrotechnical Commission (IEC) and International Telecommunication Union (ITU), are employed to ensure measurement accuracy and repeatability. The combination of these experimental techniques enables researchers to systematically study backward wave coupling, paving the way for novel device applications in signal processing, sensing, and communications.
Backward Wave Coupling in Photonic Devices
Backward wave coupling is a fundamental phenomenon in photonic devices, particularly in structures where electromagnetic waves interact with periodic or engineered materials. In essence, backward wave coupling refers to the process by which an incident wave, propagating in one direction, couples to another wave that propagates in the opposite direction—often as a result of scattering, reflection, or phase-matching conditions within the device. This mechanism is central to the operation of a variety of photonic components, including distributed Bragg reflectors (DBRs), fiber Bragg gratings, and certain types of waveguide-based filters.
The physical basis for backward wave coupling lies in the periodic modulation of the refractive index or the presence of periodic structures within the photonic device. When an optical wave encounters such a periodicity, it can satisfy the Bragg condition, leading to constructive interference of the reflected waves and efficient coupling of forward-propagating light into a backward-propagating mode. This is the principle behind Bragg gratings, which are widely used for wavelength-selective reflection and filtering in optical communication systems. The Optica (formerly Optical Society of America) and IEEE have published extensive research on the theoretical and practical aspects of backward wave coupling in these devices.
In integrated photonics, backward wave coupling is exploited to realize narrowband reflectors, distributed feedback (DFB) lasers, and wavelength-selective switches. For example, in DFB lasers, a periodic grating is embedded within the gain medium, enabling strong backward coupling that provides the necessary optical feedback for single-wavelength lasing. Similarly, in fiber Bragg gratings, the periodic modulation of the fiber core’s refractive index reflects specific wavelengths, making them indispensable for sensing and telecommunications applications. The Optica and IEEE are leading organizations in the standardization and dissemination of knowledge regarding these technologies.
Backward wave coupling is not limited to linear devices; it also plays a role in nonlinear and active photonic systems. For instance, in nonlinear waveguides, backward-coupled waves can interact with forward waves to generate new frequencies or modulate the intensity of the propagating light. The control and manipulation of backward wave coupling are thus critical for the design of advanced photonic circuits, sensors, and communication components. Ongoing research, supported by organizations such as National Institute of Standards and Technology (NIST), continues to refine the understanding and application of backward wave coupling in next-generation photonic devices.
Applications in Telecommunications and Signal Processing
Backward wave coupling is a phenomenon in which energy propagates in a direction opposite to the phase velocity within a medium or device. This effect is particularly significant in the fields of telecommunications and signal processing, where it underpins the operation of several critical components and systems. The unique properties of backward wave coupling enable advanced functionalities such as non-reciprocal signal transmission, frequency conversion, and enhanced filtering, all of which are essential for modern communication networks.
One of the most prominent applications of backward wave coupling is found in backward wave oscillators (BWOs), also known as carcinotrons. These devices exploit the interaction between an electron beam and a slow-wave structure to generate microwave and millimeter-wave signals. The backward wave nature allows for wide frequency tunability and high spectral purity, making BWOs valuable in radar systems, electronic warfare, and high-resolution spectroscopy. Organizations such as the National Aeronautics and Space Administration (NASA) and the European Space Agency (ESA) have utilized BWOs in spaceborne instrumentation for remote sensing and communication payloads.
Backward wave coupling is also fundamental in the design of directional couplers and circulators used in radio frequency (RF) and microwave circuits. These components leverage the phenomenon to achieve isolation between input and output ports, enabling duplex communication and protecting sensitive receiver circuits from high-power transmitter signals. The Institute of Electrical and Electronics Engineers (IEEE) has published numerous standards and technical papers detailing the implementation of backward wave couplers in telecommunication infrastructure.
In the realm of optical communications, backward wave coupling is harnessed in devices such as distributed feedback (DFB) lasers and fiber Bragg gratings. These components rely on the coupling of forward and backward propagating waves to achieve wavelength selectivity and stable single-mode operation, which are crucial for dense wavelength division multiplexing (DWDM) systems. The International Telecommunication Union (ITU) recognizes the importance of such technologies in its recommendations for global optical network standards.
Furthermore, backward wave coupling is instrumental in the development of non-reciprocal devices such as isolators and circulators, which are essential for preventing unwanted feedback and interference in complex signal processing chains. Research institutions and standardization bodies, including the ITU and IEEE, continue to advance the theoretical understanding and practical deployment of backward wave coupling in next-generation telecommunication systems.
Challenges and Limitations in Practical Implementations
Backward wave coupling, a phenomenon where energy propagates in the opposite direction to the phase velocity within certain engineered structures, presents unique opportunities for advanced photonic and microwave devices. However, its practical implementation is fraught with significant challenges and limitations that must be addressed for widespread adoption.
One of the primary challenges lies in the precise fabrication requirements of materials and structures that support backward wave propagation. These typically involve metamaterials or photonic crystals with negative refractive indices, which demand nanoscale accuracy in their periodic arrangements. Even minor deviations in geometry or material composition can disrupt the delicate balance required for backward wave coupling, leading to increased losses or complete suppression of the effect. This sensitivity to imperfections makes large-scale manufacturing both technically demanding and costly.
Another limitation is the inherent loss associated with the materials used. Many metamaterials rely on metallic components, which introduce significant ohmic losses, especially at optical frequencies. These losses can severely limit the efficiency and practical utility of devices based on backward wave coupling, such as negative-index lenses or compact isolators. While research into low-loss dielectric alternatives is ongoing, achieving the necessary electromagnetic response without sacrificing performance remains a major hurdle (Optica (formerly OSA)).
Bandwidth is also a critical concern. Backward wave coupling is often highly frequency-selective, with the effect occurring only within narrow spectral windows. This restricts the operational bandwidth of devices, limiting their applicability in broadband communication systems or tunable photonic circuits. Efforts to broaden the bandwidth typically involve complex multi-layered or graded-index structures, which further complicate fabrication and integration (IEEE).
Integration with existing photonic and electronic platforms poses additional difficulties. The exotic properties required for backward wave propagation are not always compatible with standard silicon photonics or conventional microwave circuit technologies. This incompatibility can hinder the seamless incorporation of backward wave devices into established manufacturing processes and system architectures (National Institute of Standards and Technology (NIST)).
Finally, stability and tunability remain open issues. Many backward wave structures are sensitive to environmental factors such as temperature, mechanical stress, or electromagnetic interference, which can degrade performance or shift operational parameters. Developing robust, tunable, and environmentally stable backward wave devices is an ongoing area of research, essential for their transition from laboratory demonstrations to real-world applications.
Recent Advances and Emerging Research Trends
Backward wave coupling, a phenomenon where energy or information propagates in a direction opposite to the incident wave, has garnered significant attention in recent years due to its implications for advanced photonic and microwave devices. Traditionally, backward wave effects were primarily associated with specialized structures such as backward wave oscillators and certain types of waveguides. However, recent advances in materials science, nanofabrication, and theoretical modeling have expanded the scope and potential applications of backward wave coupling.
One of the most notable developments is the use of metamaterials—engineered composites with tailored electromagnetic properties—to achieve and control backward wave propagation. These materials can exhibit negative refractive indices, enabling unique phenomena such as negative refraction and reverse Doppler effects. Research groups worldwide, including those at National Institute of Standards and Technology (NIST) and Oak Ridge National Laboratory (ORNL), have demonstrated backward wave coupling in both microwave and optical regimes using metamaterial structures. Such advances are paving the way for compact, efficient devices for signal processing, sensing, and nonreciprocal transmission.
Another emerging trend is the integration of backward wave coupling mechanisms into photonic integrated circuits (PICs). By leveraging periodic structures such as photonic crystals and coupled resonator arrays, researchers have achieved backward wave propagation at chip-scale dimensions. This has significant implications for on-chip isolators, circulators, and other nonreciprocal components essential for next-generation optical communication systems. Institutions like CERN and American Physical Society (APS) have highlighted the importance of these developments in recent symposia and publications.
Additionally, the exploration of topological photonics has introduced new paradigms for robust backward wave coupling. Topologically protected edge states can support unidirectional or backward-propagating modes that are immune to certain types of disorder and defects. This robustness is particularly attractive for applications in quantum information and secure communications, as emphasized by research initiatives at National Science Foundation (NSF) and collaborative projects funded by Defense Advanced Research Projects Agency (DARPA).
Looking forward, the convergence of advanced materials, precise fabrication techniques, and sophisticated theoretical frameworks is expected to further enhance the control and utility of backward wave coupling. Ongoing research is focused on expanding operational bandwidths, reducing losses, and integrating backward wave functionalities into scalable platforms, promising transformative impacts across telecommunications, sensing, and quantum technologies.
Future Prospects and Potential Technological Impacts
Backward wave coupling, a phenomenon where energy or information propagates in a direction opposite to the incident wave within a medium or device, is poised to play a transformative role in future photonic and electronic technologies. This effect, often observed in engineered structures such as metamaterials, photonic crystals, and certain waveguides, enables unique control over wave propagation, opening avenues for innovation in communication, sensing, and quantum information systems.
Looking ahead, the future prospects of backward wave coupling are closely tied to advances in material science and nanofabrication. The ability to precisely engineer materials at the nanoscale has already enabled the realization of negative refractive index materials, where backward wave propagation is a defining feature. Such materials, often referred to as left-handed metamaterials, have been demonstrated to support backward wave coupling, leading to novel effects like reverse Doppler shifts and negative refraction. These properties are being actively explored for applications in superlensing, which could surpass the diffraction limit of conventional optics, and in cloaking devices that render objects invisible to specific wavelengths of light. Research institutions and organizations such as the National Institute of Standards and Technology (NIST) and the Optica (formerly Optical Society of America) are at the forefront of these developments, providing standards, research, and dissemination of knowledge in photonics and metamaterials.
In telecommunications, backward wave coupling is expected to enhance the performance of devices like isolators, circulators, and filters, which are critical for managing signal flow and minimizing interference in optical networks. The integration of backward wave phenomena into silicon photonics platforms could lead to more compact, efficient, and reconfigurable components, supporting the ever-increasing demand for bandwidth and data rates. Furthermore, the unique dispersion properties associated with backward wave propagation are being investigated for their potential to enable slow light and enhanced nonlinear interactions, which are essential for future quantum communication and information processing systems.
The technological impacts of backward wave coupling extend to sensing and imaging as well. Devices leveraging this effect can achieve higher sensitivity and resolution, particularly in environments where conventional forward-propagating waves are limited by scattering or absorption. As research continues, collaborations between academic institutions, government laboratories, and industry leaders—such as those coordinated by IEEE and SPIE, the international society for optics and photonics—will be crucial in translating fundamental discoveries into practical technologies.
In summary, backward wave coupling stands as a cornerstone for next-generation photonic and electronic systems, with the potential to revolutionize fields ranging from telecommunications to quantum technologies and advanced imaging. Continued interdisciplinary research and standardization efforts will be key to unlocking its full technological impact.
Sources & References
- IEEE
- National Institute of Standards and Technology (NIST)
- National Aeronautics and Space Administration (NASA)
- Oak Ridge National Laboratory (ORNL)
- Nature Publishing Group
- International Telecommunication Union (ITU)
- European Space Agency (ESA)
- CERN
- National Science Foundation (NSF)
- Defense Advanced Research Projects Agency (DARPA)
- SPIE, the international society for optics and photonics