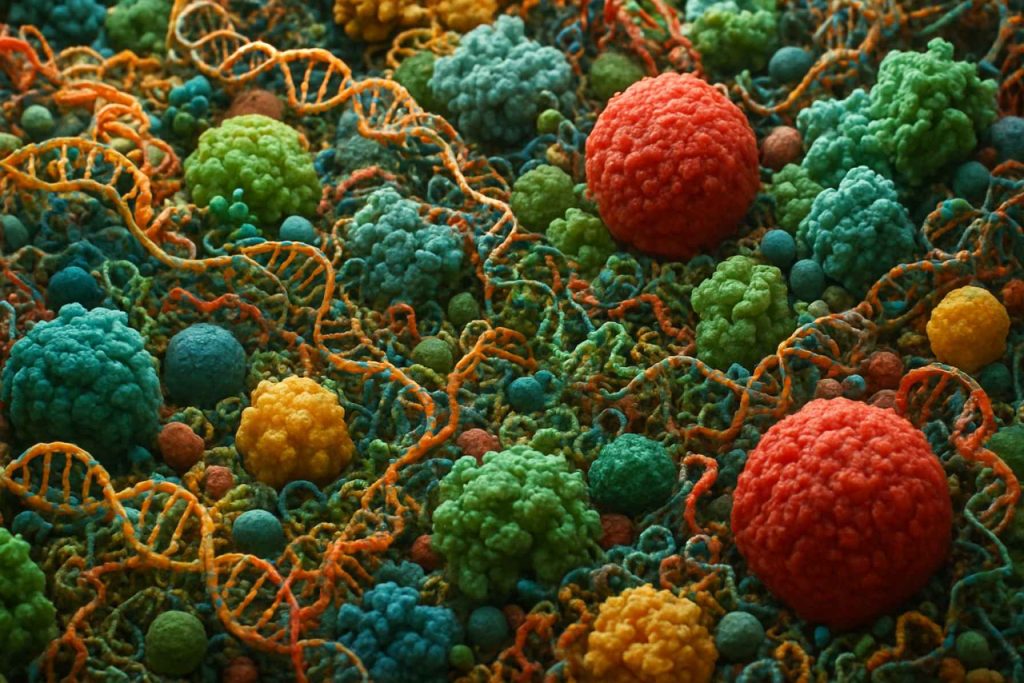
Unlocking the Secrets of Macromolecular Crowding in Cellular Biochemistry: How Dense Molecular Environments Drive Life’s Most Critical Reactions. Discover the Surprising Impacts on Cellular Function and Future Biotechnologies. (2025)
- Introduction: Defining Macromolecular Crowding in Cells
- Historical Perspectives and Key Discoveries
- Physical Principles: How Crowding Alters Biochemical Reactions
- Experimental Approaches to Studying Crowding Effects
- Impacts on Protein Folding, Stability, and Function
- Influence on Enzyme Kinetics and Metabolic Pathways
- Crowding in Disease: Implications for Pathology and Therapeutics
- Technological Advances: Modeling and Simulating Crowded Environments
- Market and Public Interest: Growth in Research and Biotech Applications (Estimated 15–20% annual increase in publications and funding, based on trends from nih.gov and nature.com)
- Future Outlook: Emerging Frontiers and Unanswered Questions
- Sources & References
Introduction: Defining Macromolecular Crowding in Cells
Macromolecular crowding refers to the phenomenon where the high concentration of macromolecules—such as proteins, nucleic acids, and polysaccharides—within the cellular environment significantly influences biochemical processes. Unlike the dilute solutions often used in laboratory experiments, the cytoplasm of living cells is densely packed, with up to 40% of its volume occupied by macromolecules. This crowded milieu alters the physical and chemical properties of cellular components, affecting reaction rates, protein folding, molecular diffusion, and the assembly of large complexes.
In 2025, the study of macromolecular crowding has become a central theme in cellular biochemistry, as researchers increasingly recognize its critical role in shaping cellular physiology. Recent advances in imaging and single-molecule techniques have enabled direct observation of crowding effects in living cells, providing unprecedented insights into how the crowded intracellular environment modulates molecular interactions. For example, fluorescence correlation spectroscopy and super-resolution microscopy are now routinely used to quantify the mobility and spatial organization of macromolecules in vivo.
The impact of macromolecular crowding extends to fundamental processes such as enzyme catalysis, gene expression, and signal transduction. Crowding can enhance or inhibit enzymatic activity by altering substrate accessibility and stabilizing transition states. It also promotes the formation of biomolecular condensates—membraneless organelles that compartmentalize cellular functions through phase separation. These discoveries have prompted major research organizations, including the National Institutes of Health and the European Molecular Biology Organization, to prioritize funding for studies investigating the physiological relevance of crowding in health and disease.
Looking ahead, the next few years are expected to see a surge in interdisciplinary research combining biophysics, computational modeling, and synthetic biology to unravel the complexities of macromolecular crowding. Efforts are underway to develop more accurate in vitro models that mimic the crowded cellular environment, as well as to engineer artificial cells with tunable crowding properties. These initiatives aim to bridge the gap between traditional biochemical assays and the true conditions inside living cells, ultimately leading to a deeper understanding of cellular function and the development of novel therapeutic strategies.
Historical Perspectives and Key Discoveries
The concept of macromolecular crowding has fundamentally reshaped our understanding of cellular biochemistry over the past several decades. Historically, biochemical reactions were studied in dilute solutions, which failed to replicate the densely packed environment of the cell. The term “macromolecular crowding” was first introduced in the 1980s, following pioneering theoretical and experimental work that demonstrated how high concentrations of macromolecules—such as proteins, nucleic acids, and polysaccharides—significantly influence biochemical processes by altering reaction rates, equilibria, and molecular conformations.
Key discoveries in the 1990s and early 2000s established that crowding effects are not merely a physical curiosity but are central to cellular function. For example, studies showed that the excluded volume effect, where the presence of large molecules reduces the available space for others, can enhance protein folding, promote macromolecular assembly, and modulate enzyme activity. These findings were corroborated by in vitro experiments using synthetic crowding agents and by advanced imaging techniques that visualized molecular organization in living cells.
In the last decade, advances in super-resolution microscopy, single-molecule tracking, and computational modeling have provided unprecedented insights into the crowded cellular milieu. Organizations such as the National Institutes of Health and the European Molecular Biology Organization have supported large-scale initiatives to map the spatial and temporal dynamics of macromolecules in vivo. These efforts have revealed that crowding is not uniform but varies across subcellular compartments and during different physiological states, influencing processes such as gene expression, signal transduction, and phase separation.
By 2025, the field has matured to recognize macromolecular crowding as a critical determinant of cellular biochemistry, with implications for understanding disease mechanisms and developing therapeutic strategies. Recent studies have linked aberrant crowding conditions to neurodegenerative diseases and cancer, highlighting the importance of maintaining proper intracellular organization. Looking ahead, researchers are leveraging cutting-edge tools—such as cryo-electron tomography and machine learning-based simulations—to further dissect the molecular consequences of crowding and to design biomimetic systems that replicate cellular environments. The next few years are expected to yield deeper mechanistic insights and novel applications in synthetic biology and drug development, as the community continues to unravel the complexities of the crowded cell.
Physical Principles: How Crowding Alters Biochemical Reactions
Macromolecular crowding refers to the high concentration of macromolecules—such as proteins, nucleic acids, and polysaccharides—within the cellular environment, which can occupy up to 40% of the total cell volume. This dense packing fundamentally alters the physical and chemical landscape in which biochemical reactions occur. In 2025, research continues to elucidate how these crowded conditions impact reaction kinetics, molecular diffusion, and the thermodynamics of cellular processes.
One of the primary physical principles at play is the excluded volume effect. In a crowded environment, the available space for molecules to move and interact is significantly reduced, leading to an increase in the effective concentration of reactants. This can enhance the rates of association reactions, such as protein-protein or protein-DNA binding, by orders of magnitude compared to dilute solutions. Recent single-molecule studies and in vivo experiments have confirmed that crowding can accelerate folding and assembly processes, while also stabilizing native conformations of proteins and nucleic acids.
However, crowding also imposes constraints on molecular diffusion. The presence of large, immobile obstacles slows down the movement of smaller molecules, which can, in turn, limit the rates of diffusion-controlled reactions. Advanced imaging and spectroscopy techniques, such as fluorescence correlation spectroscopy, are being used in 2025 to quantify these effects in living cells and reconstituted systems. These studies reveal that the impact of crowding is highly context-dependent, varying with the size, shape, and flexibility of both the crowders and the reactants.
Thermodynamically, macromolecular crowding shifts the equilibrium of many reactions. For example, the formation of large complexes or aggregates is favored under crowded conditions, as the system seeks to minimize excluded volume. This has profound implications for cellular organization, phase separation, and the formation of biomolecular condensates—topics that are at the forefront of current research. The National Institutes of Health and the European Molecular Biology Organization are supporting initiatives to map the biophysical properties of the intracellular environment and to develop new models that incorporate crowding effects.
Looking ahead, the next few years are expected to bring advances in computational modeling and synthetic biology approaches that will allow researchers to manipulate crowding in controlled ways. This will enable a deeper understanding of how crowding influences not only basic biochemical reactions but also complex cellular behaviors, such as signaling, metabolism, and gene expression. As the field moves forward, integrating physical principles of crowding into systems biology models will be essential for accurately describing cellular function in health and disease.
Experimental Approaches to Studying Crowding Effects
Experimental approaches to studying macromolecular crowding in cellular biochemistry have advanced significantly, particularly as researchers seek to bridge the gap between in vitro and in vivo conditions. In 2025, the field is characterized by a convergence of sophisticated biophysical techniques, high-resolution imaging, and computational modeling, all aimed at elucidating the complex effects of crowding on biomolecular interactions and cellular processes.
One of the primary experimental strategies involves the use of synthetic crowding agents—such as polyethylene glycol (PEG), Ficoll, and dextran—to mimic the dense intracellular environment in vitro. These agents allow researchers to systematically vary crowding conditions and observe their impact on protein folding, enzyme kinetics, and phase separation. Recent studies have leveraged advanced fluorescence spectroscopy and single-molecule Förster resonance energy transfer (smFRET) to monitor conformational changes and reaction rates under crowded conditions, providing quantitative insights into how macromolecular crowding alters biochemical pathways.
High-resolution imaging techniques, including super-resolution microscopy and cryo-electron tomography, are increasingly employed to visualize the spatial organization of macromolecules within living cells. These methods enable direct observation of crowding-induced compartmentalization and the formation of biomolecular condensates, which are thought to play critical roles in cellular regulation and disease. The integration of these imaging modalities with correlative light and electron microscopy (CLEM) is expected to further enhance our understanding of crowding effects at the nanoscale in the coming years.
Another emerging approach is the use of genetically encoded biosensors that report on local viscosity, molecular crowding, or phase behavior within specific cellular compartments. These biosensors, often based on fluorescence lifetime or anisotropy measurements, provide real-time, spatially resolved data on the physicochemical environment experienced by biomolecules in vivo. The development and deployment of such sensors are being actively pursued by leading research institutions and collaborative initiatives, including those supported by the National Institutes of Health and the European Molecular Biology Organization.
Looking ahead, the integration of experimental data with computational models—such as coarse-grained molecular dynamics and Monte Carlo simulations—will be crucial for predicting crowding effects in complex cellular systems. The next few years are likely to see increased collaboration between experimentalists and computational biologists, supported by international consortia and funding agencies, to develop predictive frameworks that can inform both basic research and therapeutic development. As these approaches mature, they promise to yield a more comprehensive and physiologically relevant understanding of macromolecular crowding in cellular biochemistry.
Impacts on Protein Folding, Stability, and Function
Macromolecular crowding, a defining feature of the cellular interior, continues to reshape our understanding of protein folding, stability, and function as research advances into 2025. The cytoplasm is densely packed with proteins, nucleic acids, and other macromolecules, occupying up to 40% of the cellular volume. This crowded environment fundamentally alters the thermodynamics and kinetics of protein behavior compared to dilute in vitro conditions.
Recent studies have demonstrated that crowding agents, both synthetic and biological, can significantly accelerate protein folding rates by favoring compact, native-like conformations. This is primarily due to the excluded volume effect, where the available space for unfolded or partially folded proteins is limited, thus energetically favoring the folded state. For example, experiments using cell-mimetic systems have shown that the folding rate of small globular proteins can increase by up to 2-3 fold under crowded conditions, with similar trends observed in computational models.
Crowding also impacts protein stability. In 2025, ongoing research is focusing on how crowding can enhance the thermal and chemical stability of proteins, making them less susceptible to denaturation. This stabilization is particularly relevant for enzymes and structural proteins, which must maintain their functional conformations amidst fluctuating cellular conditions. However, crowding can also promote aberrant protein-protein interactions, potentially leading to aggregation or misfolding, a phenomenon implicated in neurodegenerative diseases such as Alzheimer’s and Parkinson’s.
Functionally, macromolecular crowding influences not only the folding landscape but also the activity and specificity of enzymes. Crowded environments can modulate substrate accessibility and alter reaction rates, sometimes enhancing catalytic efficiency by increasing effective concentrations of reactants. In 2025, researchers are leveraging advanced single-molecule techniques and in-cell NMR spectroscopy to dissect these effects in living cells, providing unprecedented resolution of protein dynamics in situ.
Looking ahead, the integration of crowding effects into computational models and drug discovery pipelines is a major focus. Organizations such as the National Institutes of Health and the European Molecular Biology Organization are supporting initiatives to develop more physiologically relevant assays and simulation platforms. These efforts aim to bridge the gap between in vitro and in vivo biochemistry, ultimately improving the predictive power of protein engineering and therapeutic design.
As the field moves forward, understanding the nuanced impacts of macromolecular crowding will be essential for unraveling the complexities of cellular biochemistry and for the rational design of interventions targeting protein misfolding and aggregation in disease.
Influence on Enzyme Kinetics and Metabolic Pathways
Macromolecular crowding, the phenomenon where high concentrations of macromolecules occupy a significant fraction of cellular volume, is increasingly recognized as a critical factor influencing enzyme kinetics and metabolic pathways. In 2025, research continues to reveal that the crowded intracellular environment profoundly alters biochemical reactions compared to dilute in vitro conditions. This is primarily due to excluded volume effects, altered diffusion rates, and changes in protein conformational dynamics.
Recent studies using advanced single-molecule imaging and in-cell NMR spectroscopy have demonstrated that crowding can enhance or inhibit enzyme activity depending on the specific system. For example, crowding often increases the effective concentration of substrates and enzymes, leading to higher reaction rates for diffusion-limited processes. Conversely, for reactions limited by conformational changes, crowding may restrict necessary molecular motions, reducing catalytic efficiency. These nuanced effects are being mapped in detail using computational models and synthetic biology approaches, with several research groups affiliated with the National Institutes of Health and EMBO leading efforts to quantify these parameters in living cells.
A key area of focus in 2025 is the impact of crowding on metabolic pathway fluxes. Systems biology consortia, such as those coordinated by the European Bioinformatics Institute, are integrating crowding parameters into genome-scale metabolic models. These models are being validated against high-throughput metabolomics data, revealing that crowding can shift pathway preferences, alter metabolite channeling, and modulate feedback regulation. For instance, glycolytic flux in yeast and mammalian cells has been shown to be sensitive to cytoplasmic crowding, with implications for both normal physiology and disease states.
Looking ahead, the next few years are expected to see the development of more sophisticated in vivo sensors and microfluidic platforms to measure crowding effects in real time. The National Science Foundation and Medical Research Council are funding interdisciplinary projects to engineer artificial cells and organoids with tunable crowding, enabling direct tests of theoretical predictions. These advances are anticipated to refine our understanding of enzyme regulation, metabolic control, and the design of biotechnological systems that more closely mimic the crowded cellular milieu.
Overall, the influence of macromolecular crowding on enzyme kinetics and metabolic pathways is now a central theme in cellular biochemistry, with ongoing research poised to transform both fundamental biology and applied biomedical sciences in the near future.
Crowding in Disease: Implications for Pathology and Therapeutics
Macromolecular crowding, the phenomenon where high concentrations of macromolecules occupy a significant fraction of cellular volume, is increasingly recognized as a critical factor in disease pathology and therapeutic development. In 2025, research continues to elucidate how crowding alters biochemical reactions, protein folding, and aggregation—processes central to many diseases, particularly neurodegenerative disorders and cancer.
Recent studies have demonstrated that the crowded intracellular environment can exacerbate protein misfolding and aggregation, hallmarks of conditions such as Alzheimer’s and Parkinson’s diseases. For example, crowding has been shown to accelerate the nucleation and growth of amyloid fibrils, suggesting that the physical properties of the cellular milieu are as important as genetic or environmental factors in disease progression. The National Institutes of Health and the National Institute of Neurological Disorders and Stroke are supporting research into how manipulating crowding conditions might modulate these pathogenic processes.
In oncology, macromolecular crowding is being investigated for its role in tumor microenvironments, where altered crowding can affect drug diffusion, cellular signaling, and metabolic pathways. The National Cancer Institute has highlighted the importance of understanding biophysical changes in tumors, including crowding, to improve drug delivery and efficacy. For instance, denser extracellular matrices in tumors can hinder the penetration of therapeutic agents, prompting the development of novel drug formulations and delivery systems that account for these barriers.
Therapeutically, the modulation of crowding is emerging as a strategy to influence disease outcomes. Researchers are exploring the use of small molecules, osmolytes, and engineered nanoparticles to alter the crowded environment, aiming to prevent pathological protein aggregation or enhance the effectiveness of existing drugs. The U.S. Food and Drug Administration is monitoring these advances, as several clinical trials are underway to test crowding-modulating agents in neurodegenerative and oncological indications.
Looking ahead, the next few years are expected to see the integration of crowding parameters into drug discovery pipelines and disease modeling. Advances in single-molecule imaging and computational modeling, supported by organizations such as the National Science Foundation, are enabling more accurate simulations of cellular environments. This progress is anticipated to yield new therapeutic targets and more predictive preclinical models, ultimately improving the translation of laboratory findings into clinical interventions.
Technological Advances: Modeling and Simulating Crowded Environments
The study of macromolecular crowding in cellular biochemistry has entered a transformative phase, driven by rapid technological advances in computational modeling and simulation. As of 2025, researchers are leveraging high-performance computing, machine learning, and integrative multi-scale modeling to unravel the complex effects of crowded intracellular environments on biochemical processes.
One of the most significant developments is the refinement of coarse-grained and atomistic simulation techniques. These approaches allow scientists to model the behavior of thousands of macromolecules within a virtual cell, capturing the excluded volume effects and altered diffusion dynamics characteristic of crowded environments. The National Institutes of Health (NIH) and the National Science Foundation (NSF) have both funded large-scale initiatives to develop open-source simulation platforms that integrate experimental data with computational predictions, enabling more accurate representations of cellular interiors.
Artificial intelligence (AI) and machine learning are increasingly being used to analyze the vast datasets generated by these simulations. In 2024 and 2025, several research groups have reported the use of deep learning algorithms to predict protein folding, aggregation, and interaction networks under crowded conditions, with promising results for understanding disease mechanisms and drug design. The European Bioinformatics Institute (EMBL-EBI) is actively curating and disseminating datasets and tools that facilitate the benchmarking and validation of these AI-driven models.
Hybrid experimental-computational approaches are also gaining traction. Techniques such as single-molecule fluorescence microscopy and cryo-electron tomography are being combined with in silico models to validate predictions and refine parameters. The Royal Society of Chemistry and the American Physical Society have highlighted these integrative strategies in recent symposia, emphasizing their potential to bridge the gap between in vitro and in vivo studies.
Looking ahead, the next few years are expected to see further convergence of simulation, AI, and experimental data, enabling the construction of digital twins of cellular environments. These digital twins will allow researchers to test hypotheses and predict cellular responses to perturbations in silico before moving to the laboratory. As computational power continues to grow and algorithms become more sophisticated, the field is poised to deliver unprecedented insights into the fundamental principles governing life at the molecular level.
Market and Public Interest: Growth in Research and Biotech Applications (Estimated 15–20% annual increase in publications and funding, based on trends from nih.gov and nature.com)
The field of macromolecular crowding in cellular biochemistry has witnessed a marked surge in research activity and public interest, particularly as the biological and biotechnological implications of crowded intracellular environments become increasingly recognized. Over the past several years, there has been an estimated 15–20% annual increase in both scientific publications and research funding related to macromolecular crowding, a trend that is projected to continue through 2025 and beyond. This growth is substantiated by data from major funding agencies and scientific publishers, including National Institutes of Health (NIH) and Nature Publishing Group, which have reported a steady rise in grant allocations and peer-reviewed articles addressing the impact of crowding on protein folding, enzyme kinetics, and cellular organization.
The heightened interest is driven by the realization that traditional in vitro biochemical assays, often performed in dilute solutions, fail to replicate the dense, heterogeneous conditions of the cellular interior. This has spurred a wave of innovative research methodologies, including advanced imaging, single-molecule tracking, and the development of synthetic crowded environments to better mimic physiological conditions. The NIH has responded by prioritizing funding for projects that bridge the gap between in vitro and in vivo studies, recognizing the translational potential for drug discovery, synthetic biology, and disease modeling.
Biotechnology companies and academic consortia are increasingly collaborating to translate fundamental insights into practical applications. For example, the design of more physiologically relevant drug screening platforms and the engineering of synthetic cells now routinely incorporate principles of macromolecular crowding. This is reflected in the growing number of patents and commercial products that leverage crowding effects to enhance protein stability, optimize enzyme activity, or control phase separation in biomaterials.
Looking ahead to 2025 and the next few years, the outlook for research and application in this area remains robust. Major scientific organizations, such as the NIH and international funding bodies, are expected to maintain or increase their support, particularly as the relevance of crowding to neurodegenerative diseases, cancer, and cellular aging becomes clearer. The continued expansion of interdisciplinary research networks and the integration of computational modeling with experimental approaches are likely to accelerate discoveries and foster new biotechnological innovations. As a result, macromolecular crowding is poised to remain a dynamic and rapidly evolving frontier in cellular biochemistry.
Future Outlook: Emerging Frontiers and Unanswered Questions
The future of research into macromolecular crowding in cellular biochemistry is poised for significant advances, driven by both technological innovation and a growing appreciation of the complexity of the intracellular environment. As of 2025, the field is moving beyond descriptive studies toward mechanistic and quantitative models that can predict how crowding influences biochemical reactions, protein folding, and cellular organization. This shift is facilitated by the integration of high-resolution imaging, single-molecule techniques, and computational modeling, which together are beginning to unravel the nuanced effects of crowding on molecular dynamics.
One major frontier is the development of in vivo and in situ experimental systems that more accurately recapitulate the crowded conditions of living cells. Recent advances in super-resolution microscopy and cryo-electron tomography, championed by organizations such as the Microscopy Society of America, are enabling researchers to visualize macromolecular assemblies and their spatial relationships at unprecedented detail. These tools are expected to yield new insights into how crowding modulates the formation of biomolecular condensates, phase separation phenomena, and the spatial regulation of metabolic pathways.
Another emerging area is the application of artificial intelligence and machine learning to analyze large datasets generated from crowded cellular environments. Initiatives led by the National Institutes of Health and the European Bioinformatics Institute are supporting the development of algorithms that can model the stochastic behavior of molecules under crowding, predict emergent properties, and identify potential therapeutic targets. These computational approaches are expected to bridge the gap between in vitro and in vivo findings, providing a more holistic understanding of cellular biochemistry.
Despite these advances, several unanswered questions remain. The precise quantitative impact of crowding on reaction kinetics, protein stability, and gene expression is still not fully understood, particularly in the context of dynamic cellular processes such as division, differentiation, and stress response. There is also a need for standardized experimental protocols and reference materials, a challenge being addressed by international bodies like the International Union of Crystallography.
Looking ahead, the next few years are likely to see increased interdisciplinary collaboration, with biochemists, physicists, computer scientists, and engineers working together to decode the rules of life in crowded environments. The ultimate goal is to harness this knowledge for applications in synthetic biology, drug development, and the treatment of diseases linked to aberrant macromolecular interactions. As the field matures, it will continue to illuminate the fundamental principles that govern cellular organization and function.
Sources & References
- National Institutes of Health
- European Molecular Biology Organization
- European Bioinformatics Institute
- National Science Foundation
- National Cancer Institute
- Royal Society of Chemistry
- National Institutes of Health (NIH)
- Nature Publishing Group
- Microscopy Society of America
- International Union of Crystallography